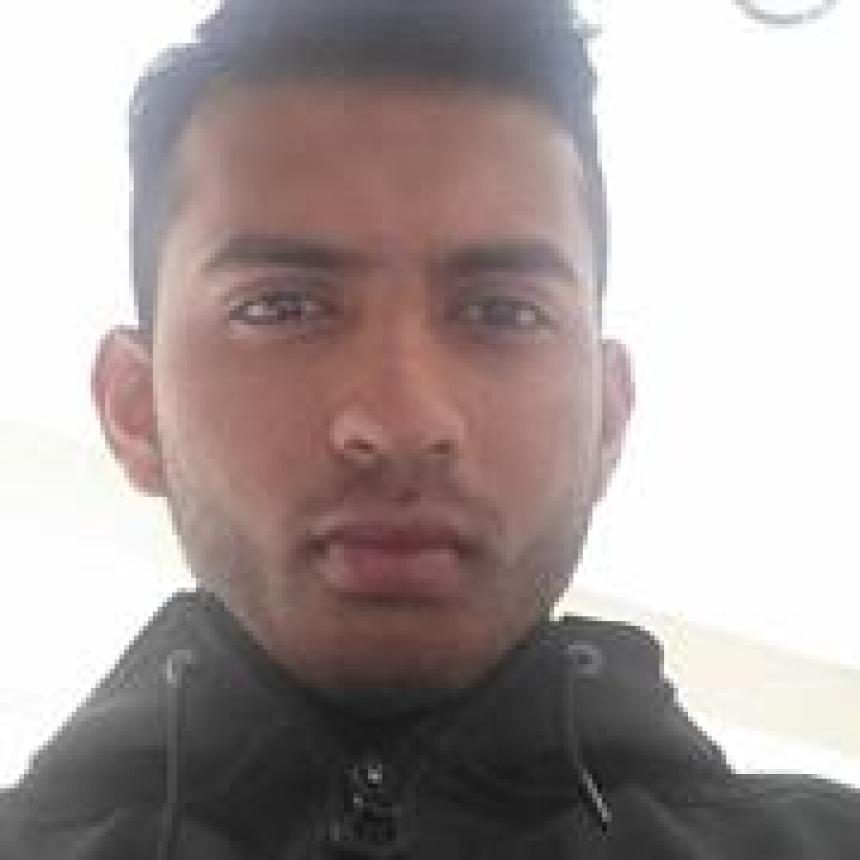
Suraj Suresh
Higher Degree by Research Candidate
School of Biomedicine
Faculty of Health and Medical Sciences
I am PhD candidate in the Brain Stimulation, Imaging and Cognition Group located in SAHMRI. My research aims to develop rTMS plasticity protocols with the goal to alter brain activity and ideally improve performance of a variety of cognitive processes (e.g. working memory). The findings from this projects will play a significant role in broadening functional use of rTMS in both a therapeutic and research related settings.
Research Q1: Can repetitive paired-pulse stimulation increase corticospinal excitability when applied to the motor cortex?
Paired pulse TMS (PPS) is a type of patterned rTMS methodology that has shown some potential to alter brain activity by efficiently targeting neuroplastic mechanisms. A seminal paper by Thickbroom et al. (2006) explored the capabilities of this protocol by using a 1.5 ms ISI to target a period of net increased cortical excitability known as an I-wave to reinforce trans-synaptic events in cortical interneurons. In this protocol, paired pulses were delivered every 5 seconds for 30 minutes at an intensity where the pair of stimuli produced a MEP of ~0.5-1 mV. This was predicted to elevate the efficacy of synaptic plasticity induction which was confirmed by a 5-fold increase in MEP amplitude during the trial which subsequently lasted for ~10 minutes post stimulation. The increase in excitability following PPS appears to be ISI dependent, with separate studies using ISIs that targeted the trough between I-wave descending volleys (e.g.3 ms) inducing a reduction in MEP amplitudes. A systematic review conducted by Kidgell et al. (2016) found that PPS targeting I-waves is an effective and safe protocol to augment brain activity within the motor cortex [20].
In addition to I-wave latencies, other temporal windows of net excitation following TMS hold potential to enhance the ability for inducing plasticity with PPS. For example, intracortical facilitation (ICF) is a period of excitability observed using a 10-15 ms ISI and subthreshold conditioning pulse. A study by Liepert et al. (1997) showed that a glutamate antagonist suppressed ICF, which suggests that the glutamatergic system plays a crucial role in the modulation of the faciliatory circuit [21]. Furthermore, repetitive PPS at an ISIs of 10-15 ms also increase MEP amplitudes in a similar way to PPS targeting I-waves [22].
Stimulation intensity plays an important role in determining the amount of net excitation observed with paired-pulse paradigms. For example, Biabani et al. (2021), found that there was a much greater increase in mean MEP amplitude when using a suprathreshold conditioning stimulus (120% of resting motor threshold, RMT) compared to a subthreshold stimulus (80% RMT) [23] at 10-15 ms ISIs. Furthermore, the period of facilitation lasted longer with a suprathreshold conditioning stimuli, extending to ISIs of 30 ms. I have conducted a further pilot analysis on the data collected by Biabani et al to assess whether MEP amplitudes were altered across trials within stimulation blocks. I found there was a significant increase in MEP amplitudes across 20 trials at certain ISIs (10, 20, 30, & 200 ms), with the largest increases observed at 20 ms (figure 1). Importantly, these increases were observed across most participants. The data from this pilot analysis introduce the fascinating possibility that suprathreshold PPS could rapidly increase corticospinal excitability by inducing neuroplasticity. However, the current data only observed changes in excitability within stimulation blocks, and it remains unclear whether the increases in excitability last beyond the period of stimulation.
Research Question 2: Can audio masking optimise data collection during TMS-EEG?
MEPs are effective in measuring how rTMS paradigms alter cortical excitability over motor cortex. However, there are significant issues that limit our ability to form a complete understanding of the paradigm's efficacy. One major problem with this approach is we can only elucidate how each paradigm alters brain activity within the motor cortex. In other words, we can’t use MEPs to measure plasticity outcomes following stimulation of other brain regions. Another issue is that MEPs don’t necessarily define the degree of change that is occurring in the brain, since there is a strong possibility of spinal contributions altering MEP amplitude, making it difficult to determine the extent of the changes which are occurring in cortical regions.
However, there are other methodologies that can help circumvent these issues. One approach is to use electroencephalography (EEG) to record the brain’s response to single pulse TMS [24]. EEG measures the electrical activity of the brain across time, and thus provides a direct measure of the cortical response to TMS which does not include contributions from the spinal cord. Furthermore, EEG allows researchers to record the brain’s response from stimulation to any cortical region, not just the motor cortex. As such, TMS-evoked potentials (TEPs) provide an excellent alternative to MEPs for indexing changes in cortical excitability following rTMS. This was illustrated in a study by Chung et al. (2017) who used TMS-EEG to determine the effects of iTBS and cTBS on the dorsal lateral prefrontal cortex and successfully elucidated that both paradigms were able to modulate cortical reactivity as observed by changes in TEP amplitudes [25].
While TMS-EEG holds promise as an effective technique to determine how NIBS effects brain activity following rTMS protocols particularly from non-motor regions, there are still limitations associated with TMS-EEG which can impact interpretation of the data. One problem is the presence of artifacts, which represent activity that doesn't originate directly from the stimulated brain region [24]. For example, a study by Nikouline et al. (1999) showed that both bone and air-conducted clicking sounds associated with generation of the TMS pulse resulted in auditory-evoked potentials which masked TMS-evoked potentials, posing as a serious contaminating factor significantly compromising the data acquisition process [24]. As a result, studies often apply some kind of noise masking like playing white noise through headphones to try and prevent auditory-evoked potentials following TMS. While this approach was widely believed to be successful, several recent studies have shown this method often is insufficient to prevent auditory-evoked potentials [26, 27]. In addition to white noise, other studies have suggested using a masking noise made up of phase shuffled signals derived directly from the TMS clicking noise. However, it remains unclear whether these alternative auditory masking approaches can further reduce auditory-evoked potentials following TMS.
Research Question 3: Can suprathreshold PPS alter brain dynamics in non-motor regions to alter working memory performance?
Investigating how rTMS protocols augment cortical excitability within the motor cortex has provided an important foundation for understanding how TMS manipulates brain activity through plasticity. However, rTMS is often applied to non-motor regions to investigate the role of these regions in cognitive tasks, or as a therapy for brain disorders. For example, a number of studies have targeted the dorsolateral prefrontal cortex with rTMS to assess the role of this region in a cognitive process known as working memory (WM). WM is a cognitive process termed by Miller et al. (1968) which describe the ability to store and manipulate information in the mind for brief periods (e.g., several seconds) [28, 29]. Several brain regions appear important for WM function, including the dorsolateral prefrontal cortex (DLPFC), anterior cingulate cortex (ACC) and the parietal cortex (PAC). The DLPFC is particularly important in executive control and influences other brain regions to determine how information is integrated during WM, and may also play a role in storing information. Subsequently, studies have targeted the DLPFC with rTMS to further define the functional characteristics of this region, and determine whether rTMS over DLPFC is able to reliably improve WM performance [30-32].
The current consensus concerning how NIBS alters WM performance is equivocal. A meta-analysis by Brunoni et al. (2014) determined that the majority of rTMS studies applied to the DLPFC resulted in significant improvements in working memory performance [33]. However, the overall effect sizes were small (Hedge’s g ~0.2-0.3). A series of studies aimed to further explore this phenomenon using TMS-EEG to measure changes in cortical excitability as well as working memory performance following iTBS over DLPFC. A study by Hoy et al. (2015) showed that applying iTBS to the DLPFC significantly improved WM performance, while also improving oscillatory brain activity associated with WM [34]. However, a study by Chung et al. (2018) ran a similar experiment but weren’t able to replicate the results, finding a high level of inter-individual variability in both TMS-EEG and behavioural outcomes. In a follow up study, Chung et al. (2019) attempted to improve outcomes by tailoring the iTBS protocols to the participant's physiology by varying frequency based on neurophysiological markers derived from EEG. This approach appeared beneficial for both TMS-EEG and behavioural measures, however effects sizes were still small [35] [36] and the TMS-EEG measures were likely highly contaminated by auditory-evoked potentials. Consequently, exploring other avenues of stimulation such as suprathreshold PPS may provide more reliable changes and robust alteration in cortical excitability and WM performance based on the data acquired in the pilot study
-
Appointments
Date Position Institution name 2021 - ongoing PhD candidate University of Adelaide -
Education
Date Institution name Country Title 2019 - 2020 Monash University Australia Masters of Biomedical and health sciences 2015 - 2018 Flinders University Australia Bachelors of science (animal behaviour) (honours) -
Research Interests
-
Journals
Connect With Me
External Profiles